AIA course number: BE2022A; GBCI course #: 920025449
Credit Type(s): 1 AIA LU/HSW; 1 IIBEC CEH; 0.1 IACET CEU; 1 GBCI CE Hour
Learning Objectives:
After reading this article, you should be able to:
- Define sustainability.
- Evaluate operational carbon, embodied carbon, and whole life carbon footprint of buildings.
- Discuss how roofing contributes to sustainability goals.
- Integrate holistic system design approaches to roofing overburden.
Complete the quiz and receive a certificate of completion!
Introduction
Sustainability is too often seen as an afterthought to how we design and construct buildings. However, if we shift our thinking to a more holistic approach to not only the building itself but how it interacts with its surrounding environment and community, and use collaborative design from the start of the design process, the carbon footprint can be minimized, and limiting global Greenhouse Gas (GHG) emissions to 1.5 degrees Celsius can be achieved.1
Commercial roofs can be “benefit multipliers” by integrating renewable energy, rainwater management strategies, and rooftop agriculture to address our changing environment and more severe storms.
The intent of this course is to discuss the interconnectedness and interrelations of roof strategies that can help minimize whole building carbon emissions while, at the same time, improve long-term performance, durability, and resiliency by using overburden strategies to alleviate the community burden of climate change.
Let’s Start with a Definition
In 1987, the United Nations Brundtland Commission defined sustainability as “meeting the needs of the present without compromising the ability of future generations to meet their own needs.”2 While this definition can be interpreted as overly simplistic and too broad, it highlights the key ingredient of sustainable development: time. It is specifically the temporal component that we as humans seem to struggle with. We tend to create a false dichotomy between short-term gains and long-term impacts. However, sustainability is not asking us to sacrifice our needs today, quite the opposite. It is explicitly telling us that we should meet our needs today; however, we must not get bogged down in short-term thinking and keep an eye on the future to ensure future generations are not jeopardized by the decisions we make now.
The Earth is Warming: IPCC Report
The climate data in the most recent International Panel on Climate Change (IPCC) report makes it evident that the Earth is warming and the effects of climate change are already being felt. The IPCC 2021 report states, “It is unequivocal that human influence has warmed the atmosphere, ocean and land. Widespread and rapid changes in the atmosphere, ocean, cryosphere and biosphere have occurred.”3
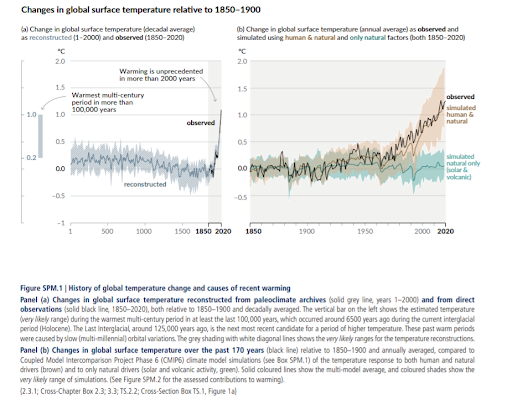
In the U.S., catastrophic weather events such as severe storms, flooding, drought, heat waves, and wildfires have become more frequent and more costly. As NOAA National Centers for Environmental Information (NCEI) reported in January 2022,
“In 2021, the U.S. experienced 20 separate billion-dollar weather and climate disasters, putting 2021 in second place for the most disasters in a calendar year, behind the record 22 separate billion-dollar events in 2020. What really made 2021 stand out was the diversity of disasters:
- 1 winter storm/cold wave event (focused across the deep south and Texas);
- 1 wildfire event (combined impacts of wildfires across Arizona, California, Colorado, Idaho, Montana, Oregon and Washington);
- 1 drought and heat wave event (summer/fall across western U.S.);
- 2 flood events (in California and Louisiana);
- 3 tornado outbreaks (including the December tornado outbreaks);
- 4 tropical cyclones (Elsa, Fred, Ida and Nicholas); and
- 8 severe weather events (across many parts of the country, including the December Midwest derecho).”4
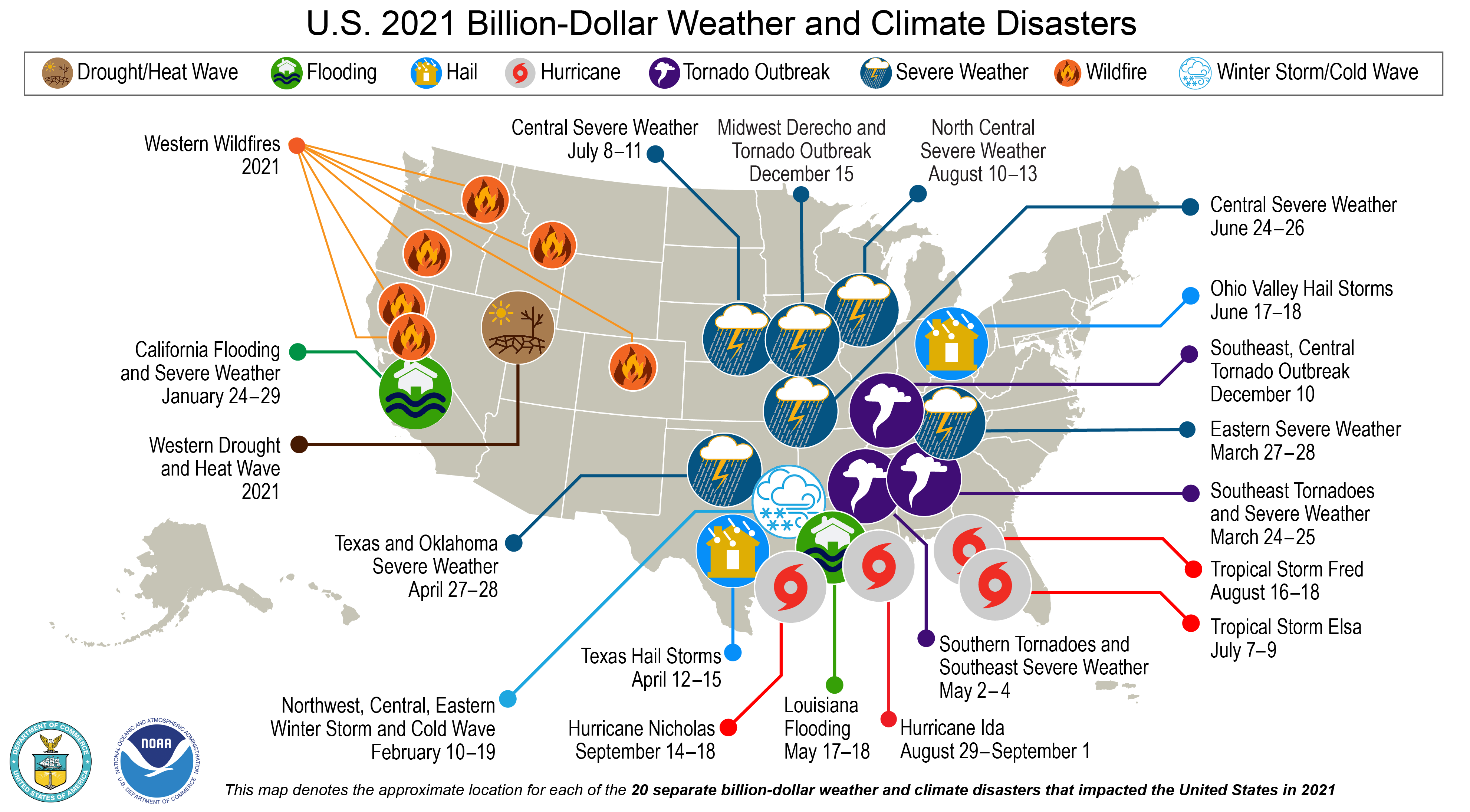
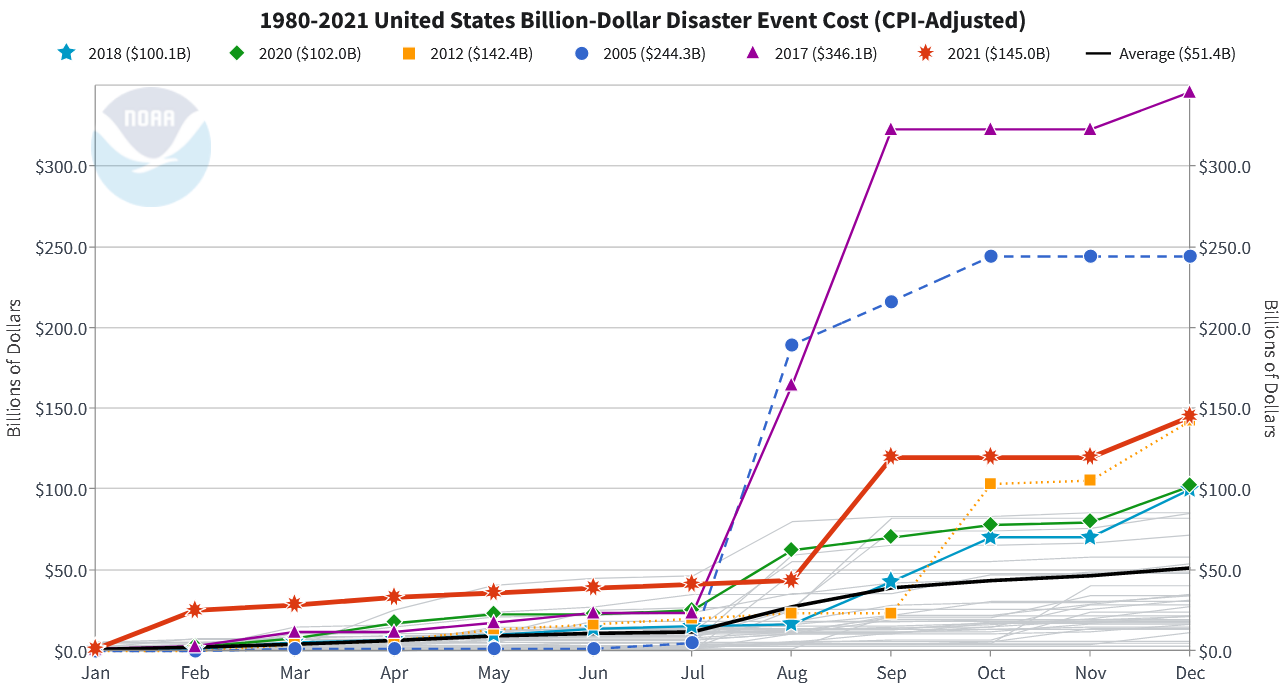
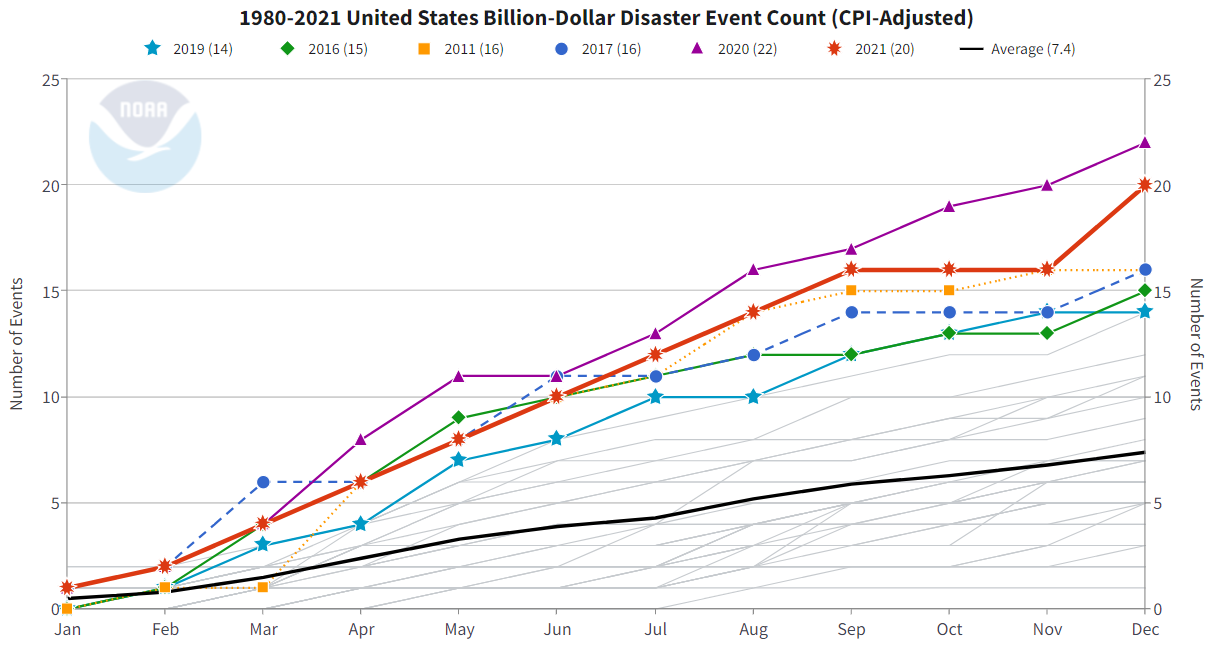
Indeed, climate change is here. So what can we do about it? As the IPCC report recommends, “From a physical science perspective, limiting human-induced global warming to a specific level requires limiting cumulative CO2 emissions, reaching at least net zero CO2 emissions, along with strong reductions in other greenhouse gas emissions. Strong, rapid and sustained reductions in CH4 emissions would also limit the warming effect resulting from declining aerosol pollution and would improve air quality.”5
So, we need to limit CO2 emissions to reach net zero emissions; or, in other words, decarbonize human activities on Earth. In addition, emissions of methane (CH4), another potent greenhouse gas, should be limited and reduced. How methane relates will be discussed later as we evaluate source energy, renewables and embodied carbon.
How much time do we have? The IPCC estimates that we have approximately 10-30 years to limit global warming to 1.5C (2.7 degrees Fahrenheit) and minimize the most catastrophic effects of climate change.6 It is worth noting that this is based on current rates of emissions; so, continuing with “business as usual” gives us 10 years at best, while significantly reducing emissions immediately will provide more time to limit warming. Although this may seem impossible, our built environment is the best driver and has the greatest potential impact to solve the climate crisis and save not only ourselves, but also future generations. By using a holistic approach to understanding how building systems are interrelated to total building energy use, while at the same time interconnected with the larger energy infrastructure ecosystem and community, building professionals have the unique opportunity and the responsibility to affect positive change to preserve the planet for human habitation.
It’s Not Too Late—Architecture 2030
At the 2021 United Nations Climate Change Conference, more commonly known as COP26, Ed Mazria from Architecture 2030 delivered a road map on how the built environment can reach zero emissions by 2040. If CO2 emissions are reduced 65 percent by 2030, there is a better than 67 percent probability that zero emissions can be met by 2040.7 As Mark C. Pederson noted in 2020, “The news about real action on climate change tends to track toward the gloomy. It is easy to despair, given the severity of the problem and the time left to properly address it. But there is progress being made in the built environment—just not nearly fast enough to offset emissions elsewhere. In recent years the sector has added billions of square feet of new buildings, but seen energy consumption for the entire sector actually decline.”8
In other words, building energy efficiency measures are working and we have already decoupled emissions from building energy use. This is a cause for optimism, as this has been accomplished even as the population continues to grow. One of the main contributors to efficiency improvements over the last decade has been building enclosure upgrades that use more robust continuous insulation, air tightness measures, and—the opposite side of the same coin—more efficient mechanical systems for ventilation, heating and cooling. While it’s tempting to give all the credit to the natural gas transition and renewable energy installations, the impact of high efficiency building enclosures cannot be underestimated. On the other hand, we need to do better and we can do better. By going beyond code minimums and using holistic energy efficiency design strategies for all new construction, net-zero operational emissions can easily and cost effectively be met today. However, the main obstacle to accomplish this at scale is not a technological issue, it’s a labor issue. In order to reduce the building sector’s emissions to meet Architecture 2030’s goals, it must be done rapidly and at scale. The construction industry requires a massive workforce development and training program on how to build and retrofit existing buildings using energy efficiency design principles. This workforce development applies not only to younger generations, but also to older workers who need new skills where their industries have become obsolete. An added benefit to scaling workforce development for energy efficient construction is that construction jobs tend to be localized which keeps money in the local community, creates other local businesses to support the construction sector, and provides a more resilient local economy that is buffered from global market fluctuations in an increasingly volatile stock market.
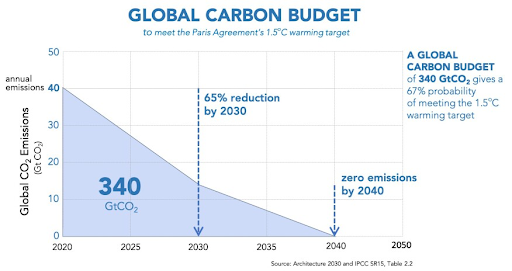
It is commonly thought that buildings account for approximately 30-40 percent of GHG emissions. However, as the chart below shows, when we factor in the embodied carbon, or “... the greenhouse gas emissions arising from the manufacturing, transportation, installation, maintenance, and disposal of building materials of building materials and construction,” buildings account for nearly half of global emissions.9 If we look at urban centers like New York City, over 70 percent of emissions come from building operations, (i.e, heating, cooling, and plug loads).10 In addition, approximately 83 percent of the U.S. population lives in cities.11 So, it follows that fixing the urban built environment can have the greatest impact to realize climate action goals and preserve the welfare of the most people. As noted earlier, this is no small task and requires a collaborative holistic approach as well as workforce training and mobilization at a scale not seen since World War II.

While there is a role for government in incentivizing a rapidly scaling market transformation, the private sector is the main driver in affecting this change. In fact, some manufacturers already have trade training programs to educate the unemployed, the underemployed, military veterans, and the formerly incarcerated at a scale to meet the labor shortages that already exist in the construction industry.12 It is not a huge leap to incorporate energy efficiency principles into these types of training programs.
With many U.S. states and car manufacturers setting goals for 100 percent electrification by 2035, transforming the built environment has the unique capability to significantly reduce or eliminate emissions from the transportation sector. This, however, requires a shift from the traditional way of thinking about buildings as static, standalone units to a dynamic part of a larger interconnected community infrastructure ecosystem. Every building has the potential to generate more energy than it uses. By integrating rooftop solar, electric vehicle charging infrastructure, and battery storage into energy efficient building designs, a large part or all of the transportation sector emissions can potentially be eliminated. This not only helps reduce emissions but also reduces transmission losses from delivering electricity over long distances and creates a more resilient localized energy grid to minimize power outages from the aging large scale electric infrastructure.
So What Does This Have to Do with Roofing?
The primary purpose of roofs is to provide shelter from the elements. However, roofs are the often unseen work horses of our buildings. Roofs already do a lot of heavy lifting, both literally and figuratively. Whether it’s providing protection against wind, rain, snow and hail, or reflecting solar rays, supporting rooftop mechanicals, minimizing fire risk, providing daylighting, and insulating—roofs humbly endure for decades.
This “out of sight and out of mind” perception of roofs is changing rapidly as the population grows and becomes increasingly urbanized in a warming world. In New York City, Local Laws 92 & 94 have already been enacted and require that, “The sustainable roof must include a solar photovoltaic system generating at least 4kW, a green roof system, or a combination of the two. Vertical or horizontal enlargements must also comply with these requirements.”13 In addition to the solar and vegetated roof mandate in NYC, “Local Law 94 increases the SRI (solar reflectance index) of cool roofs to keep up with LEED v4. The law also adds new reflectance requirements for sloped roofs. Coatings are readily available, inexpensive and easy to apply.”14
Similar laws and ordinances exist in various cities across the U.S. For example, Seattle has the Seattle Green Factor, a landscaping requirement that green roofs can help meet.15 In addition, Seattle is also requiring roofs to be solar energy ready.16 Washington, D.C. has rebates for vegetated roofs to help manage rainwater, and initiatives for rooftop solar.17,18
Roofs now may need to incorporate vegetation, rainwater capture, rainwater retention/detention, and solar arrays. In addition, roofs are now being used for urban agricultural purposes, biodiversity restoration, and much needed outdoor greenspace in cities with high population densities. Roofs are a climate benefit multiplier that sustainability professionals and the AEC community alike should be collaborating on to develop strategies and solutions for.
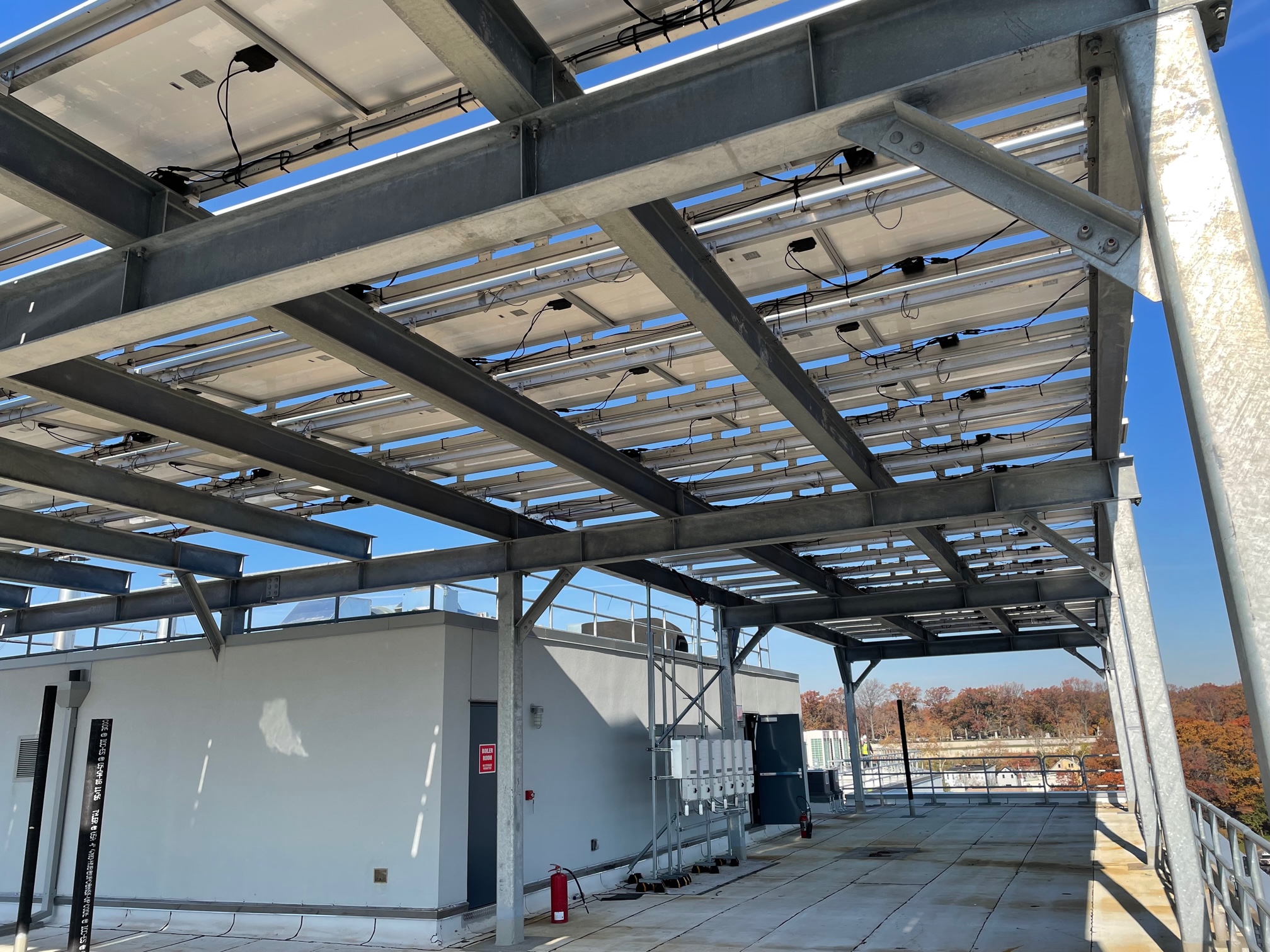
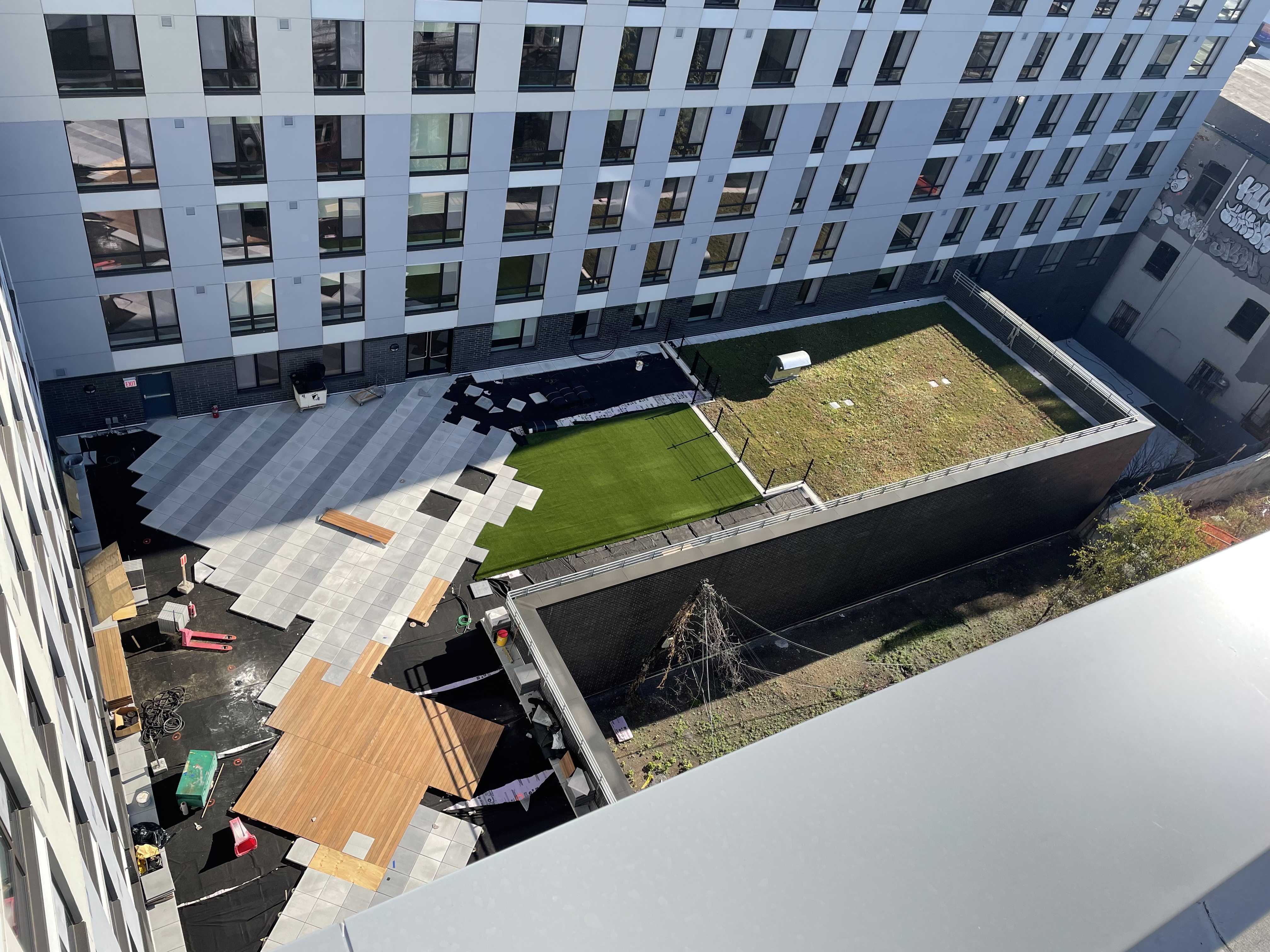
Embodied, Operational and Total Carbon
A holistic “total carbon” understanding and analysis is essential to decarbonizing the built environment. A paradigm shift away from the traditional segmented, compartmentalized, or—to use corporate speak—“siloed” way of designing, building, and doing business is essential to minimizing the effects of climate change. Using collaborative design from the start and encouraging scope creep is essential to understanding the interrelatedness and interconnections of the decisions we make. A holistic design approach viewed through the lens of “whole life carbon” is necessary to future-proof the built environment. “Business as usual” will only continue to provide the usual outcomes.
Operational Carbon
According to the World Green Building Council, net zero operational carbon building is a “highly energy efficient building with all remaining energy from onsite and/or offsite renewable sources.”19 From an operational point of view, this means the building has reduced energy demand and consumption to a low enough point that all of its energy needs can be provided by renewable energy.
Factors that influence the operational efficiency of a building from a building enclosure standpoint include continuity of insulation, airtightness, thermal bridges, fenestrations and—the opposite side of the same coin—air handling unit size and efficiency. Careful detailing and integration of the roof membrane (or vapor retarder) into the vertical wall air control layer and continuous thermal envelope with climate specific effective R-values should be evaluated as part of the complete building enclosure. Effective R-values, not nominal R-values, that include heat losses (or gains) from thermal bridges, should all be included and analyzed with holistic building energy models. Code minimum values may or may not be sufficient to meet the energy efficiency needs of the building and require a deep understanding of the interrelationships of building systems to find the most optimal energy balance between heat losses and heat gains. The Solar Reflectance Index (SRI) and not only its contribution to the energy efficiency of the building, but also how it relates to the urban heat island effect and the community at large should also be considered.
While lighting, plug loads and domestic hot water are also important to consider, they are variables mostly controlled by occupant behavior and have been left out of the discussion for the building enclosure focus of this article.
Passive design strategies, or the performance-driven Passive House Standard, are highly effective and have been well documented globally over the past 30 years. As Michael Tobias, the Founding Principal of NY Engineers recently noted, “A key benefit of passive house design is being able to heat and cool buildings at a very low cost, without affecting comfort. When a building is designed with these principles, it consumes 75-95 percent less energy than a traditional building.”20 However, one should be careful to not confuse “Passive House” with the “Passive Solar” movement of the 1970’s and 80’s. Modern passive houses, derived from the German “passivhaus,” are neither fully passive nor only for single family houses. The Passive House Standard applies to every building typology in every climate globally, including but not limited to schools, hospitals, prisons, offices, multi-unit residential, manufacturing, and single family homes. The key difference between passive solar and passive house is the addition of balanced heat recovery ventilation (HRV) or energy recovery ventilation (ERV). By reducing the energy demand for heating and cooling by 75-95 percent (as Tobias suggests) and transitioning to all electric mechanicals, the remaining energy consumption can easily and cost effectively be met by onsite renewables or community solar.

Thermal Bridging
“Thermal bridges are localized areas of high heat flow through walls, roofs, and other insulated building envelope components. Thermal bridging is caused by conductive elements that penetrate and/or bypass thermal insulation, and/or misaligned planes of thermal insulation. These paths allow heat flow to bypass the insulating layer, and reduce the effectiveness of the insulation.”21 As building enclosures become more insulated and more air tight, the effects of thermal bridging have a bigger impact on heat energy loss. In addition, thermal bridges can become locations of condensation which cause other moisture related issues such as conditions for mold growth and corrosion of building components.
Thermal bridges in roofing include, but are not limited to: parapets, mechanical fasteners, pipe penetrations, curbs, railings and structural components. Mechanical fasteners alone can contribute up to a 35 percent reduction or higher in effective R-values of roof assemblies.22 Strategies to reduce or eliminate thermal bridges include adhered roof assemblies, structural thermal breaks, and encapsulation of thermal bridges in insulation.
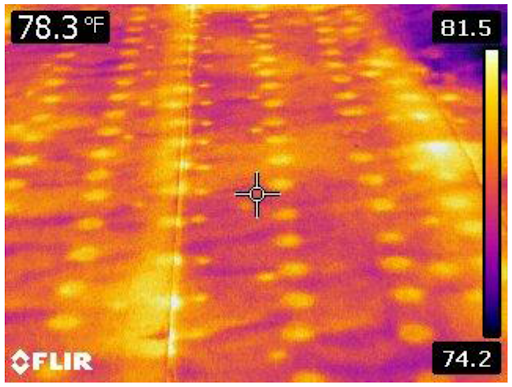
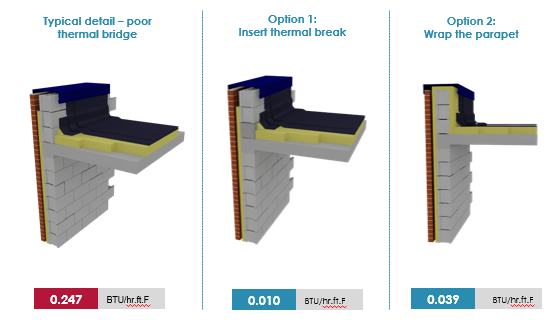
Embodied Carbon
Net zero embodied carbon, also called embodied energy, is a “building (new or renovated) or infrastructure asset [that] is highly resource efficient with upfront carbon minimized to the greatest extent possible and all remaining embodied carbon [is] reduced or, as a last resort, offset in order to achieve net zero [carbon] across its lifecycle.”23 In other words, the building materials and construction methods have utilized little or no fossil fuel energy sources during extraction, manufacturing processes, transportation, installation, maintenance, and disposal of building materials. In addition, aligned with the IPCC recommendations and as a co-benefit to reducing carbon, methane emissions from natural gas, coal and landfills are eliminated and/or minimized, with those primary energy sources being replaced by renewable energy.
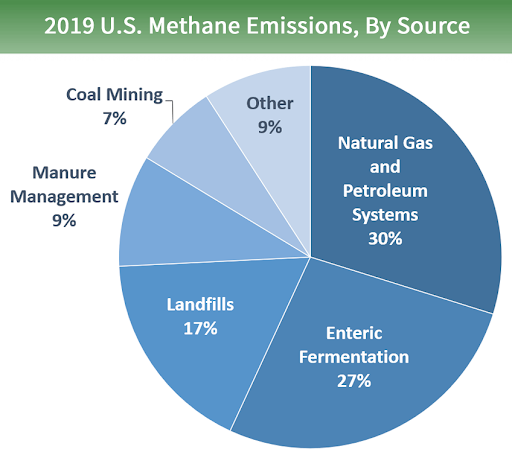
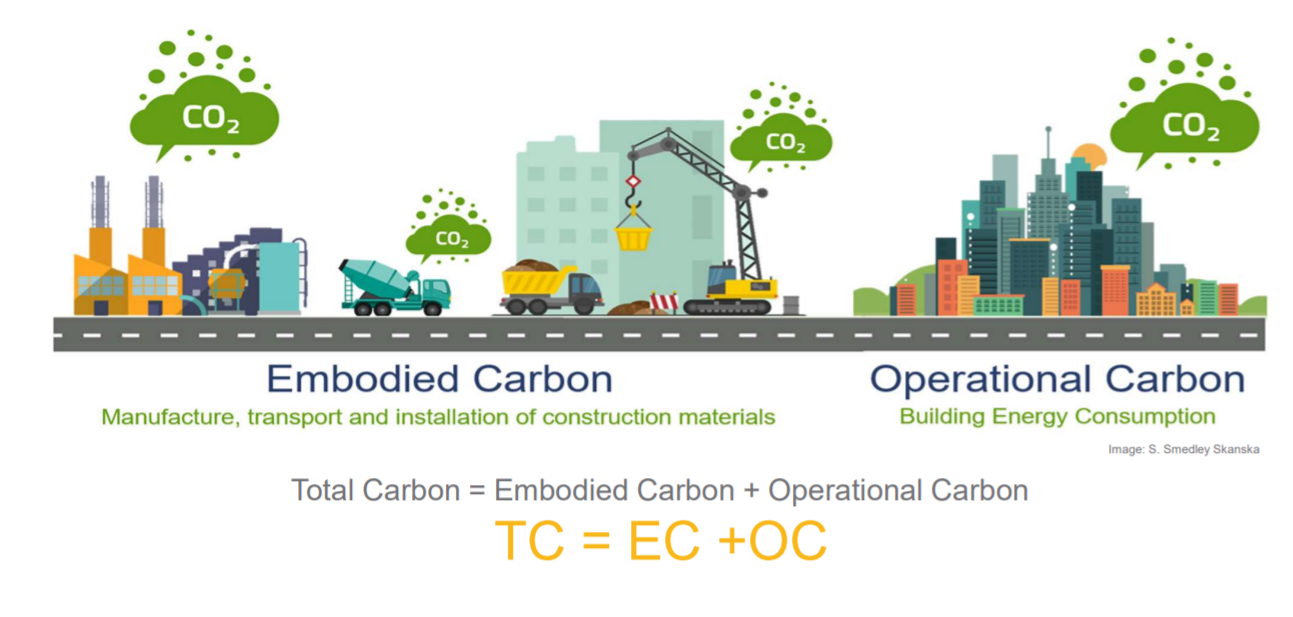
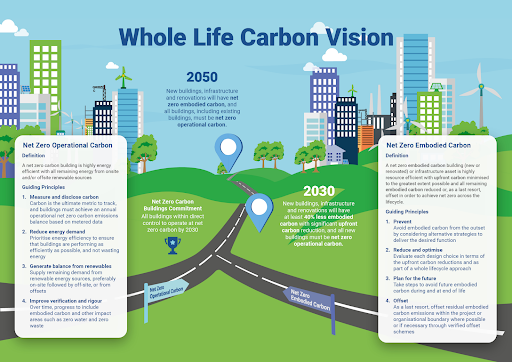
How Do We Measure Embodied Carbon?
Environmental Product Declarations (EPDs) are the best way to evaluate and compare embodied carbon of materials. EPD’s act like the MPG sticker on a new car. However, it's not always easy to compare EPD’s as they are not usually an “apples to apples” comparison, which can lead to inaccurate selection bias. In addition, EPD’s do not include operational carbon and only apply to the manufacturing and disposal phases of a product; nor do they include the replacement costs of carbon at the end of a product's useful life. For example, one type of roof may need to be replaced every 20-30 years while others can last significantly longer. Similarly, mechanicals may need to be replaced every 17-20 years. EPDs only account for the first carbon cost of a material or product. While huge improvements are being made to building material product transparency thanks to organizations like the International Living Future Institute (ILFI) and their Declare Label program to document manufacturing locations and end of life options, it doesn’t tell the full whole life carbon footprint story. See examples of an EPD and a Declare Label below.
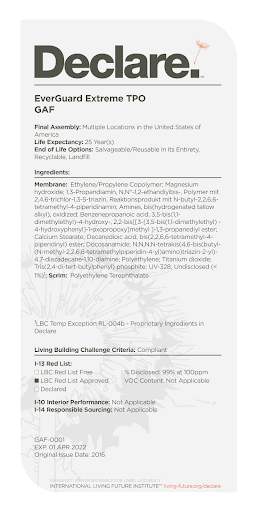

End of Life Options
According to the Ellen MacArthur Foundation,
“The circular economy is a systems solution framework that tackles global challenges like climate change, biodiversity loss, waste, and pollution. Furthermore, the circular economy is based on three principles, driven by design:
- Eliminate waste and pollution
- Circulate products and materials (at their highest value)
-
Regenerate nature
It is underpinned by a transition to renewable energy and materials. A circular economy decouples economic activity from the consumption of finite resources. It is a resilient system that is good for business, people and the environment.”24
In other words, if construction waste can be eliminated by recycling, “upcycling,” reusing, or as feedstock for biological systems, the embodied carbon footprint can be minimized or eliminated. This “closed loop” thinking requires a paradigm shift in how we think about industrial products. While it may seem counterintuitive, a shift towards circular thinking can allow us to develop new uses for building materials derived from petrochemicals that have less of an environmental impact than so-called “natural” materials. For example, a recently released study published in the Proceedings of the National Academy of Sciences looking at ethanol biofuel found that, “ethanol is likely at least 24 percent more carbon-intensive than gasoline due to emissions resulting from land use changes to grow corn, along with processing and combustion.”25 This illustrates the importance of a holistic carbon approach using science to overcome our own biases for seemingly counterintuitive truths.
At first glance asphaltic products in roofing may seem like a poor choice from an environmental standpoint. However, when those products are reclaimed at their end of life and recycled into new durable products, the initial embodied carbon is lessened vs. virgin material. In fact, significant strides are already being made by roofing companies that are developing processes to recycle up to 90 percent of torn off asphalt shingles to be used in new shingles or in asphaltic roads.26
In addition, emerging biological technologies like asphalt digesting mycelium are showing promise in new ways to handle petrochemical waste.27 Only by viewing materials from a circular viewpoint can we accurately analyze materials. However, we also need to be careful not to be influenced by savvy greenwashing marketing campaigns and the allure of “wishcycling” as have been commonplace in the plastics industry.28 Only understanding the total whole life carbon footprint of building materials using a science-based approach gives us the clarity to make responsible decisions.
Temporal Considerations of Embodied and Operational Carbon
According to the American Institute of Architects California, “embodied carbon emissions are released during the process that begins with sourcing materials and ends with the completion of construction; operating carbon emissions—from heating, cooling, lighting, and plug loads—occur over the life of a building, which can be 50 years or more. For new buildings, embodied carbon emissions typically equal about 20 years of operating emissions. When looking at total greenhouse gas emissions for new buildings built over the next ten years—the critical period for action to address the global climate emergency—Architecture 2030 estimates that 80 percent will come from embodied emissions, so lowering embodied carbon emissions is now even more urgent than lowering operating emissions” when operational carbon trends toward zero.29
In the short term, embodied carbon emissions have an oversized impact on total emissions. Because the embodied carbon cannot be amortized over the total life of the building (50-75 years) there is not enough time to benefit from the operational carbon savings. However, embodied carbon should not be considered in a vacuum. Operational carbon also needs to be considered for the whole life of the building. Considerations such as product lifespan, durability, and serviceability must also be considered. For example, while it is known that concrete and steel account for the majority of embodied carbon emissions in building construction, until we have better technologies to sequester carbon in materials, create a closed loop circular economy to recycle all building materials, and/or convert all manufacturing source energy to renewable energy, concrete and steel remain the best materials for long-term durability and resilience to more severe and more frequent weather events in a warming climate.
A recent study entitled “Carbon Footprint of Green Roofing: A Case Study from Sri Lankan Construction Industry” by Malka Nadeeshani et al performed a comparative study on a concrete flat roof to a concrete flat roof with a green roof, “The results further revealed that the life cycle carbon emissions of the intensive green roof are 84.71 percent lower compared to the conventional concrete flat roof. Hence, this study concludes that the use of green roofs is a suitable alternative for tropical cities for improving the green environment with substantial potential for carbon emission reduction throughout the life cycle of a building.” And, “The results revealed that the operational phase has the highest contribution to the carbon footprint of both roof types. In the operational phase, the green roof was found to significantly reduce heat transfer by nearly 90 percent compared to the concrete flat roof.”30
By adding vegetative roofs to concrete roof decks, carbon can significantly be reduced. In addition, new approaches to reducing the embodied carbon in materials like concrete are already underway. In April 2021, the UCLA CarbonBuilt project received funding to further study CO2 sequestration in concrete. “The UCLA CarbonBuilt approach captures CO2 generated during production and then infuses it into the concrete, using hydrated lime. The process uses 60 to 90 percent less ordinary portland cement (aka calcium silicate cement), and it doesn’t require as much heat, which has an energy efficiency benefit. CarbonCure also fixes CO2 into concrete. Its equipment (roughly the size of a microwave) is installed for free, but the company using it needs to purchase CO2 elsewhere. Right now, that CO2 is food-grade, but the idea is that it eventually could be collected anywhere.”31
Reducing the embodied carbon of concrete is not some far off future technology. New mixes such as Portland-Limestone Cement (PLC), also known as Type IL, are “engineered with a higher limestone content than portland cement to reduce the carbon footprint of concrete by about 10 percent. It performs just like the cement you’re used to using, resulting in the same concrete you’re used to having. The same specifications, the same mix design, now with a better carbon profile.”32
According to another study, “Using biomass fly ash as a secondary cementitious material can reduce the carbon footprint of concrete by 40 percent while maintaining good technical and environmental performance.”33
Carbon Unification—Whole Life Carbon
Whole life carbon is calculated by adding the embodied carbon emissions to the operational carbon emissions to find the total carbon emissions. In order to analyze whole life carbon, a holistic approach using collaborative design from the very start of the design process is required.
As Daniel Overbey has illustrated previously in Building Enclosure magazine, “An ideal whole-building embodied carbon modeling workflow begins at the earliest stages of design as part of an integrative design process.”34
Analyzing commonly used insulation types serves as another example of the balancing act required to properly evaluate whole life carbon. In the chart below is the operational carbon of insulation at increasing thicknesses over 30 years in Climate Zone 5 using gas heat as the primary energy source.
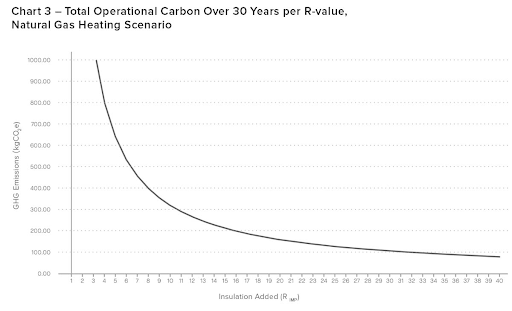
We often hear about the law of diminishing returns of insulation. While it is true that the benefits of adding insulation have less impact on energy savings at a certain point over time, this view is too simplistic. If a holistic performance based approach to the building enclosure is taken in lieu of a prescriptive approach, it is evident that insulation is just one part of an enclosure system. Air tightness, orientation, fenestration performance, thermal bridges, and building occupancy all must be considered in determining the “right-sized” insulation thickness that is specific to the climate where the building resides. Furthermore, the transition to all electric buildings supplied by renewable energy would flatten the operational emissions to zero. However, it should be noted that the building enclosure must still be optimized from an energy efficiency (operational carbon) perspective in order to minimize electric energy consumption and provide the most possible energy back to the grid or battery storage.
Now, keeping in mind what was discussed earlier regarding the oversized short term impact of embodied carbon on whole building carbon, let’s look at the embodied carbon of common insulation types. As different insulation types have different R-values/inch, the best way to standardize the embodied carbon analysis is by R-value. As R-value is directly linked to the energy efficiency of the building, and thereby the operational carbon; evaluating the embodied carbon per R-value gives insight into the interrelationship between the two.
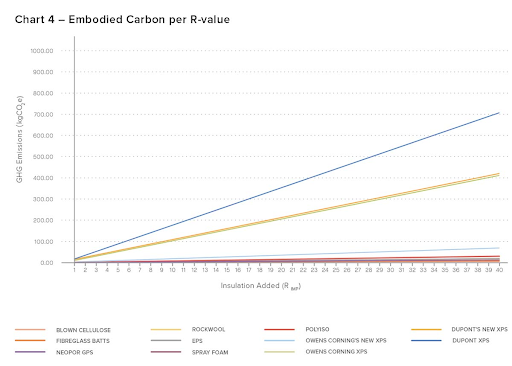
By using embodied carbon as the lens to evaluate various insulation types, designers can accurately evaluate the true impact of product selection decisions. Embodied carbon analysis allows us to cut through our own implicit biases and corporate greenwashing to have the greatest immediate impact in reducing up front carbon emissions in the built environment.
Tools for Whole Life Carbon Analysis
While there are a multitude of life cycle assessment (LCA) tools to evaluate the embodied carbon of materials, including Athena, eTool, OneClick LCA, and Tally using EC3 data from the Carbon Leadership Forum (CLF), merging both embodied and operational carbon into one whole building calculator to comprehend the full carbon picture has been an obstacle. However, as reported in Treehugger in January 2022, PHribbon, a new plugin tool for the Passive House Planning Package (PHPP), allows Passive House designers to calculate whole life carbon emissions for Passive House buildings. According to the U.S.-based Passive House Network, "This add-on enables Passive House designers to calculate the embodied carbon of a given design within the Passive House Planning Package (PHPP), an easy-to-use planning tool for energy efficiency. Integrated with the Embodied Carbon in Construction Calculator (EC3), PHribbon gives users unparalleled power to forecast the carbon emissions impact of their designs."35 What is promising about this new tool is that it incorporates all the “in-use” phase embodied carbon emissions into the whole lifecycle of the building, as well as operational carbon by taking into account the use, maintenance, repair, replacement, and refurbishment carbon costs of the building. (See B1-B5 in Figure 14.) In addition, to accommodate future data on the “Beyond Life” aspect of the building materials; reuse, recovery, and recycling potential are also included. (See D in Figure 14.) Only by understanding the interplay of all of these aspects of embodied and operational carbon can we objectively analyze whole life carbon. Whole life carbon must be measured to be managed.

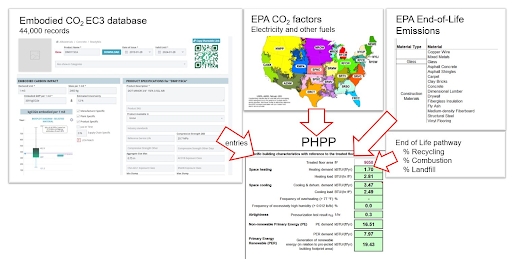
Site Energy vs. Source Energy (Primary Energy)
Understanding the difference between site energy and source energy (or primary energy) is often confusing. Fortunately, through a collaborative effort between ILFI and the Passive House Institute (PHI), a more holistic view is emerging. “Primary Energy (PE) is the total amount of raw fuel that is required to operate a building. In addition to the energy consumed on-site as the building is used, Primary, or Source Energy, includes losses that take place during generation, transmission, and distribution of the energy. PE conversion factors differ for different energy carriers (e.g. electricity or natural gas) and countries. Calculating the PE demand enables an assessment of raw fuel resource needs resulting from building operations based on the energy supply structure.”36
Furthermore, PHI has a renewable energy conversion factor to calculate how much renewable energy is needed to meet the energy demand of a building.
“To calculate the Primary Energy from Renewables (PER) demand of a building, PER conversion factors are applied as multipliers to the Site Energy. These factors differ depending on the end use (e.g. heating, cooling, hot water), the energy carrier (e.g. electricity or natural gas from renewables), and the region. They take into account storage losses caused by the variability in alignment between regional renewable generation capacity and energy demand for a specific use over the seasons of a year. For generated renewable energy, one kWh is defined as equivalent to one kWh of PER (i.e. PER factor = 1 for renewable energy supply.)”37
Overburden for Resilient Design: Integrated Roof Design as a Climate Impact Benefit Multiplier
As defined by the Resilient Design Institute, “Resilience is the capacity to adapt to changing conditions and to maintain or regain functionality and vitality in the face of stress or disturbance. It is the capacity to bounce back after a disturbance or interruption.”38 As illustrated previously, climate change related disasters are increasing in frequency and intensity. Buildings need to be prepared to manage not only the extreme weather related events but also the power outages and the human health and life safety risks that come along with them. Passive energy efficient design strategies ensure that tenants will be able to shelter in place for extended periods without power and not suffer the health-related consequences of extreme heat or extreme cold. By focusing on energy-efficiency first, then adding site renewables and battery storage, tenants may have little interruption to their normal daily activities. Furthermore, if low embodied carbon considerations are included in the energy efficient design, the cause of climate change is simultaneously being addressed.
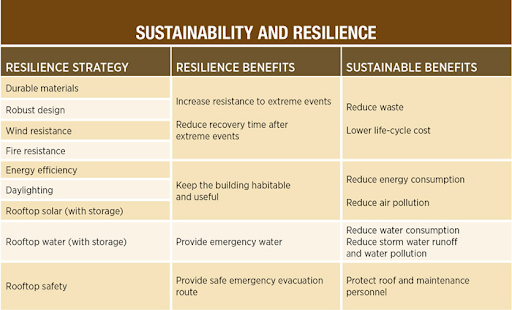
“Overburden” can be defined as any manner of material, equipment or installation that is situated on top of, and covering all or a portion of, a roof or waterproofing membrane assembly. This excludes thermal insulation but includes, and is not limited to,
- Planters, inclusive of everything they contain;
- Vegetative assemblies in trays, mats or other similar containers;
- Loose growing media, gravel, sand or any other granular material;
- Non-structural water features, inclusive of the water;
- Void fill;
- Tiles, pavers, supporting pedestals or other similar materials;
- Equipment and/or installations, other than those mounted on curbs or structural mounts which are permanently waterproofed with the roof membrane assembly39
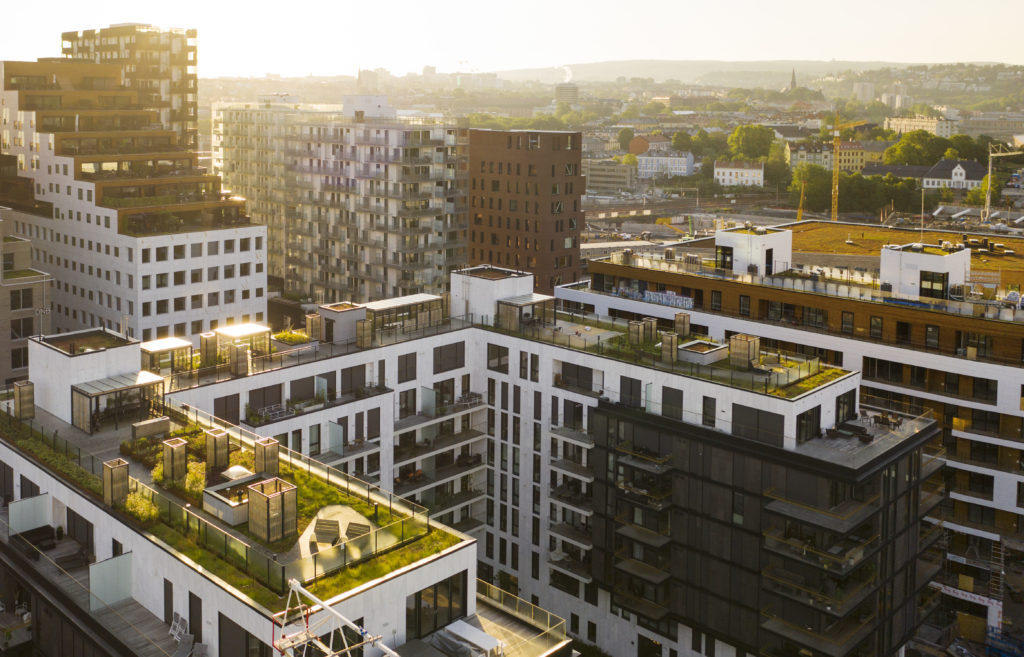
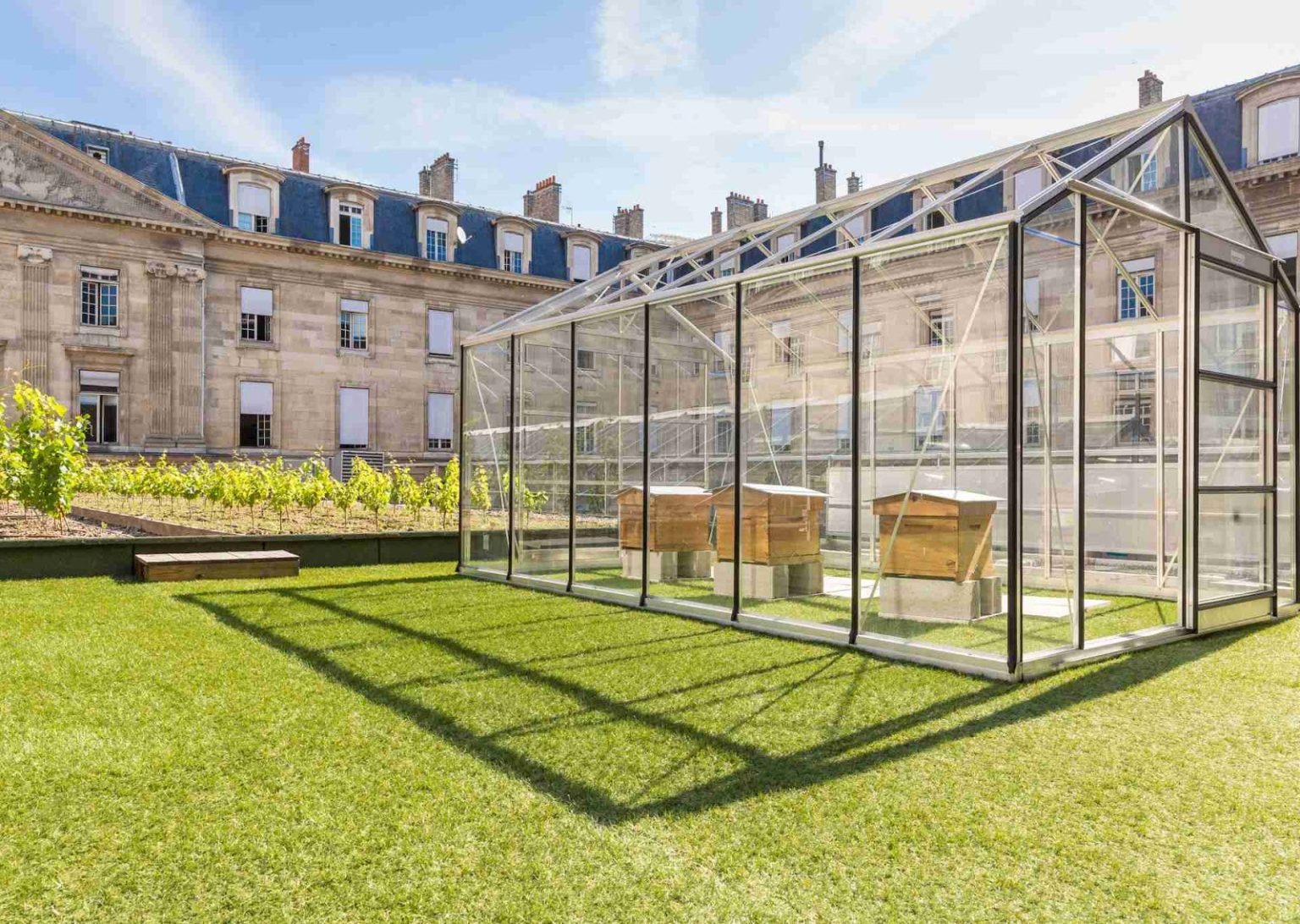
Benefits of Vegetated Roofs
The benefits of vegetated roofs, are too numerous to discuss here. However, how they relate to carbon reduction goals and building resiliency include: waste diversion and extending life of roof membranes, rainwater management, energy efficiency, increased biodiversity, and urban agriculture. First, vegetated roofs can potentially reduce waste to landfill by incorporating recycled materials into the growing medium; while, at the same time extending the useful life of membrane from the roofing membrane by protecting it from expansion/contraction stresses from temperature extremes and ultraviolet light degradation. Second, vegetated roofs can help manage rainwater by storing water in the substrate for the plants to use.
This retention of rainwater helps not only to reduce the volume of water flowing onto impervious surfaces and into the sewer system, but also increase the time it takes to runoff; thereby reducing the strain on aging sewer infrastructure. Third, vegetated roofs provide additional insulation on top of the required code minimum R-value. Fourth, vegetated roofs can provide much needed biodiversity restoration in urban areas, which may also reduce the urban heat island effect. Fifth, vegetated roofs can help reduce the ambient temperature on rooftops, thereby aiding solar arrays to function more efficiently and produce more energy. Lastly, vegetated roofs can provide local agriculture in urban “food deserts.”40 In addition, the emerging practice of agrivoltaics, or agriculture combined with photovoltaics, is showing promise as a symbiotic solution to not only increase the efficiency of solar panels, but also increase crop yields by shading and limiting soil evaporation.41
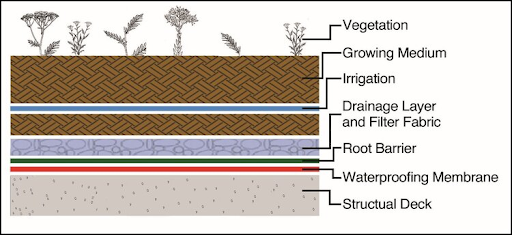
A report by New York City’s Urban Green Council notes, “Green roofs absorb rainwater and give buildings an extra layer of insulation, which can keep indoor spaces more comfortable, improve energy efficiency and lower carbon footprints. The added vegetation improves local air quality and promotes biodiversity. It also reduces the urban heat island effect, which can raise summer temperatures by up to 22F due to: (1) heat absorbed by building materials and roads; and (2) waste heat from energy use and transit.”42 And, a “50-90 percent reduction in rainwater runoff after installing a green roof.”43
Again, this is not some far off future technology. It is already being done. In fact, on the top of Manhattan’s Javits Center, a 6.75-acre sedum green roof—one of the largest in the U.S.—is now home to 29 bird species, five bat species and thousands of honeybees, and is currently seeing installation of a solar array.44 The “Javits Center opened a one-acre working farm and fruit orchard that should annually yield 40,000 pounds of produce, starting next year.”45
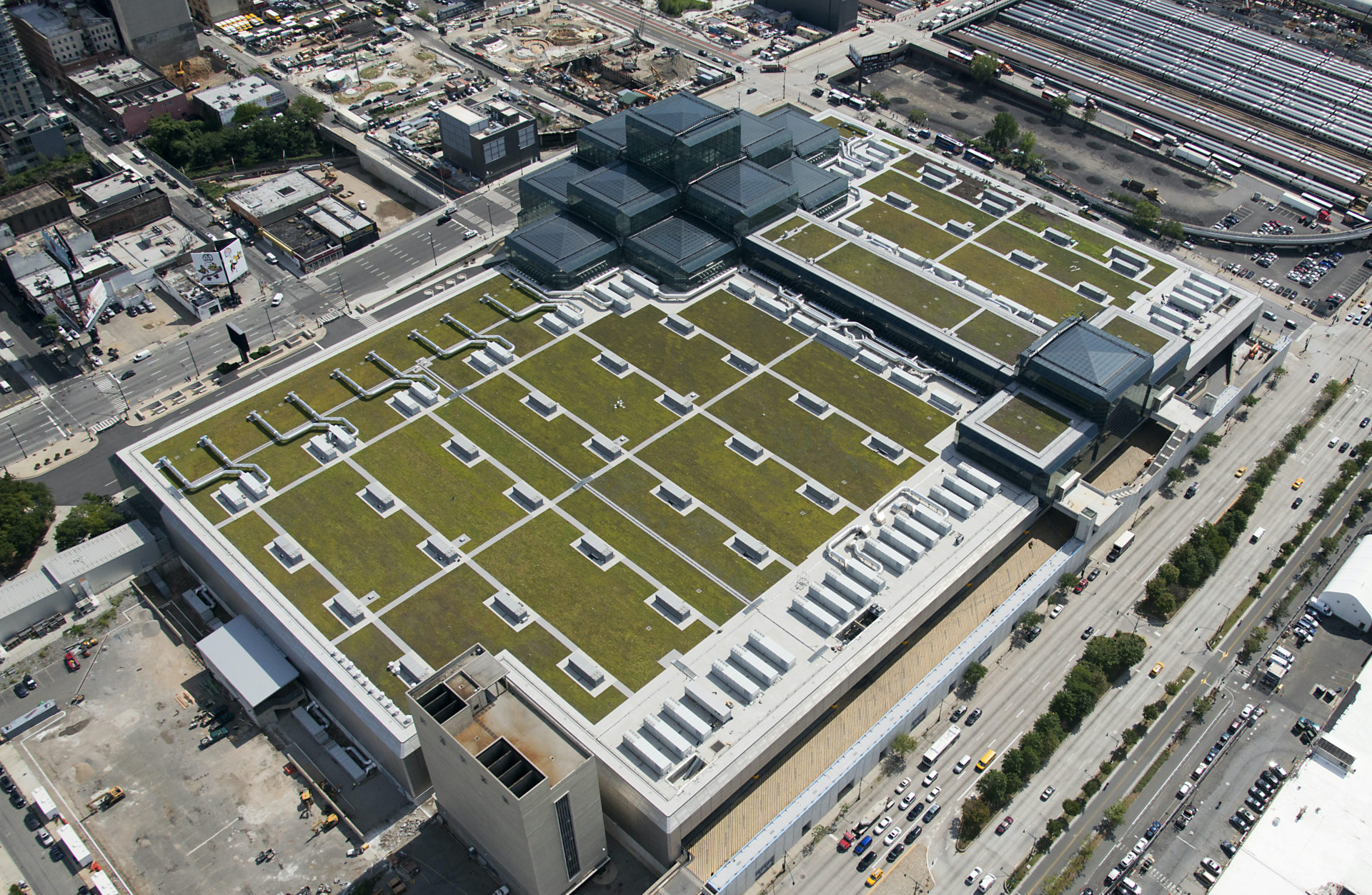

Blue, Green-Blue, and Purple Roofs
Beyond urban agriculture and clean energy generation, rooftops are now also improving on the rainwater management capabilities of vegetated roofs with “blue roof,” “blue-green roof,” and “purple roof” advancements. A blue roof is defined as “a roof designed for the retention of rainwater above the waterproofing element of the roof. This is as opposed to more conventional roofs which allow for rainwater to drain from the roof. Blue roofs are typically flat, without any fall, with control devices to regulate drainage outlets that enable water to be retained or drained.”46
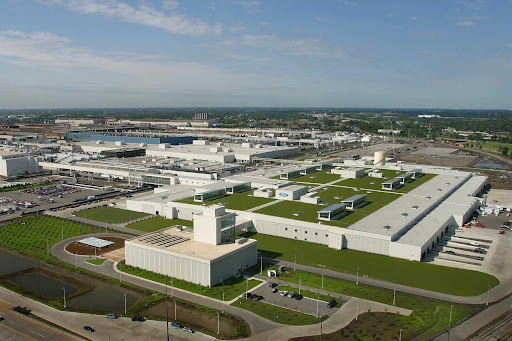
A blue-green roof is a blue roof with a vegetated roof assembly, as well. The added vegetation acts as a biological sponge to reduce the amount of rainwater runoff while, at the same time, slowing the rate at which rainwater runs off. Or, in other words, “The blue green roof combines blue and green roof technologies. Conventional green roofs use a drainage layer to provide lateral drainage and irrigation. Blue roof technology, however, aims to increase both the volume of water stored and control the amount of water released. Combining the technologies can increase the overall benefits of greening roof scapes. Therefore, it is not a case of green or blue infrastructure but a blending of the both.47
A purple roof is a blue-green roof that also incorporates a sponge-like layer made of hydrophilic mineral wool to increase the volume of rainwater that can be retained or detained and reduces peak outflow by up to 95 percent.48
Section detail of a vegetated roof. Image courtesy of GAF/Siplast Embodied Carbon AIA.
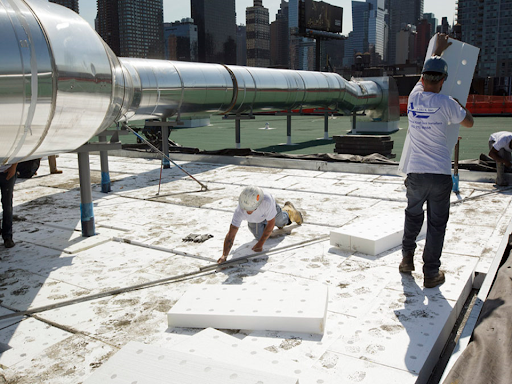
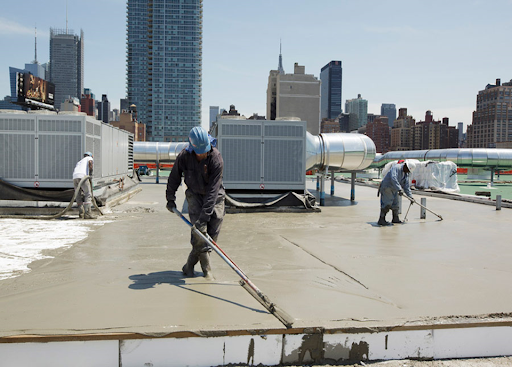
Conclusion
In summary, carbon is the unifying metric to understand sustainability and resiliency, which are all interconnected and interrelated. A holistic understanding of our building design decisions can only be understood through the lens of whole life carbon analysis. High CO2 levels in the atmosphere are causing us to develop resiliency strategies while reducing carbon in the atmosphere is the solution. It's the chicken AND the egg.
Taking all of this into account, commercial roofing has the unique opportunity as a sustainability benefit multiplier to not only shelter us from increasingly severe weather events, but also allow communities to thrive in the face of climate change, while at the same time addressing the need to reduce carbon emissions in the atmosphere. However, this is no small task. All stakeholders must work together collaboratively if we’re to realize a world where future generations can meet their own needs without compromising the needs of society today.
End Notes
1Architecture 2030. “Accelerating to Zero by 2040!” Oct. 2019. Web. 22 Feb. 2022. <https://architecture2030.org/accelerating-to-zero-by-2040/>
2https://www.un.org/en/academic-impact/sustainability Accessed 2/4/2022 at 9:33am.
3Intergovernmental Panel on Climate Change. “Climate Change 2021: The Physical Science Basis Working Group I: Contribution to the Sixth Assessment Report of the Intergovernmental Panel on Climate Change.” Oct. 2021. Web. 4 Feb. 2022. <https://www.ipcc.ch/report/ar6/wg1/downloads/report/IPCC_AR6_WGI_SPM_final.pdf>
4National Oceanic and Atmospheric Administration (NOAA). “2021 U.S. billion-dollar weather and climate disasters in historical context.” 24 Jan. 2022. Web. 6 Feb 2022. <https://www.climate.gov/news-features/blogs/beyond-data/2021-us-billion-dollar-weather-and-climate-disasters-historical#:~:text=In%202021%2C%20the%20United%20States,least%20%241%20billion%20in%20damages>
5Intergovernmental Panel on Climate Change. “Climate Change 2021: The Physical Science Basis Working Group I: Contribution to the Sixth Assessment Report of the Intergovernmental Panel on Climate Change.” Oct. 2021. Web. 4 Feb. 2022. <https://www.ipcc.ch/report/ar6/wg1/downloads/report/IPCC_AR6_WGI_SPM_final.pdf>
6Headline Statements. Intergovernmental Panel on Climate Change. Web. 8 Feb. 2022. <https://www.ipcc.ch/sr15/resources/headline-statements/>
7Architect Magazine Staff and Mazria, Edward. “Architecture 2030's Open Letter to the UN Framework Convention on Climate Change Calls for Revised Carbon Emission Reduction Targets.” Architect Magazine, 6 Nov. 2020. Web. 18 Feb. 2022. <https://www.architectmagazine.com/technology/architecture-2030s-open-letter-to-the-un-framework-convention-on-climate-change-calls-for-revised-carbon-emission-reduction-targets_o>
8Pedersen, Martin C. “Edward Mazria With Some Good News About Combating Climate Change.” “Common Edge,” 27 July 2020. Web. 9 Feb. 2022. <https://commonedge.org/edward-mazria-with-some-good-news-about-combating-climate-change/>
9Carbon Leadership Forum. “Embodied Carbon 101.” 17 Dec. 2020. Web. 8 Feb. 2022. <https://carbonleadershipforum.org/embodied-carbon-101>
10NYC Mayor’s Office of Climate & Environmental Justice. “Energy Benchmarking: Energy map.” New York City Energy & Water Performance Map.” Web. 8 Feb. 2022. <https://www1.nyc.gov/site/sustainability/codes/energy-benchmarking.page#:~:text=Over%2070%20percent%20of%20New,on%20energy%20and%20water%20management>
11Center for Sustainable Systems, University of Michigan. 2021. "U.S. Cities Factsheet." Pub. No. CSS09-06. Web. 8 Feb 2022. <https://css.umich.edu/factsheets/us-cities-factsheet#:~:text=It%20is%20estimated%20that%2083,to%20live%20in%20urban%20areas>
12Saulsbery, Gabrielle. “Peak Demand: Parsippany-based GAF addresses a serious labor shortage by training new roofers.” NJBiz. 17 May 2021. Web. 17 Feb. 2022. <https://njbiz.com/peak-demand-gaf-roofer-training/>
13La Rocca, Melanie E. “Service Notice: Local Law 92 of 2019 and Local Law 94 of 2019: Green and Solar Roof Requirements for New Buildings and Complete Roof Replacements.” NYC.gov. Oct. 2019. Web. Feb 9 2022. <https://www1.nyc.gov/assets/buildings/pdf/green_roof_solar_ll92-n-94of2019_sn.pdf>
14Urban Green Council and The Nature Conservancy. “NYC’S SUSTAINABLE ROOF LAWS.” Urban Green Council. Dec. 2019. Web. 10 Feb. 2022.
15Torgelson, Nathan. “Seattle Green Factor.” Seattle.gov. Web. 18 Feb. 2022. <https://www.seattle.gov/sdci/codes/codes-we-enforce-(a-z)/seattle-green-factor#:~:text=You%20can%20choose%20from%20a,or%20create%20a%20food%20garden>
16Seattle Department of Construction & Inspections. “Renewable Energy and Solar-Ready Roofs for Commercial Buildings.” Seattle.gov. 18 Oct 2021. Web. 14 Feb 2022. <http://www.seattle.gov/DPD/Publications/CAM/Tip422.pdf>
17Washington, D.C. Department of Energy & Environment. “Green Roofs in the District of Columbia.” Web. 22 Feb. 2022. <https://doee.dc.gov/greenroofs>
18Washington, D.C. Department of Energy & Environment. “Solar Initiatives.” Web. 22 Feb. 2022. <https://doee.dc.gov/service/solar-initiatives>
19World Green Building Council. “Whole Life Carbon Vision.” WorldGBC.org. Web. 4 Feb. 2022. <https://www.worldgbc.org/advancing-net-zero/whole-life-carbon-vision>
20Tobias, Michael. “Green Building Trends: Pros & Cons of Passive House Construction.” NewYork Engineers. 12 October 2021. Web. 17 Feb. 2022. <https://www.ny-engineers.com/blog/green-building-trends-pros-cons-of-passive-house-construction>
21Taylor, T.J. “Eliminating Fastener Thermal Bridging in Low Slope Roofs: Energy Efficiency Savings versus Installation Costs.” Open Journal of Energy Efficiency. 9, 94-110. June 2022. Web. 20 Feb. 2022. <https://doi.org/10.4236/ojee.2020.92007>
22Ibid.
23World Green Building Council. “Whole Life Carbon Vision.” WorldGBC.org. Web. 4 Feb. 2022. <https://www.worldgbc.org/advancing-net-zero/whole-life-carbon-vision>
24Ellen MacArthur Foundation. “What is a circular economy?” Web. 17 Feb 2022. <https://ellenmacarthurfoundation.org/topics/circular-economy-introduction/overview>
25Reuters. “U.S. corn-based ethanol worse for the climate than gasoline, study finds.” Autoblog. 21 Feb. 2022. Web. 2 Feb 2022.
26GAF Roof Views. “Welcome to the Future of Asphalt Recycling.” GAF.com. 21 April 2021. Web. 17 Feb 2022. <https://www.gaf.com/en-us/blog/welcome-to-the-future-of-asphalt-recycling-281474980090173>
27MYCOCYCLE. Web. 22 Feb 2022. <https://mycocycle.com/>
28Acaroglu, Leyla. “The Trap of Wishcycling.” Disruptive Design. 8 Sep. 2021. Web. 22 Feb. 2022. <https://medium.com/disruptive-design/the-trap-of-wishcycling-6ea083646876>
29Siegel, Henry and Strain, Larry. “Embodied Carbon: What you Can Do Right Now.” AIA California. 5 March 2020. Web. 8 Feb. 2022. <https://aiacalifornia.org/embodied-carbon-definitions-and-facts/>
30Nadeeshani, Malka et al. “Carbon Footprint of Green Roofing: A Case Study from Sri Lankan Construction Industry.” Sustainability 2021, 13, 6745. 15 June 2021. <https://doi.org/10.3390/su13126745>
31Clancy, Heather. “Carbon-sucking concrete is capturing attention and funding.” GreenBiz. 6 May 2021. Web. 17 Feb. 2022. <https://www.greenbiz.com/article/carbon-sucking-concrete-capturing-attention-and-funding>
32PLC-Portland Limestone Cement. “Portland-Limestone Cement U.S. Fact Sheet.” Greener Cement. Web. 17 Feb. 2022. <https://www.greenercement.com/_files/ugd/f3d485_bf593dd744f049d98cbd293109fc42ce.pdf>
33Tosti, Lorenzo et al. “Technical and environmental performance of lower carbon footprint cement mortars containing biomass fly ash as a secondary cementitious material.” Resources, Conservation and Recycling. Volume 134. July 2018. <https://doi.org/10.1016/j.resconrec.2018.03.004>
34Overbey, Daniel. “Defining a Whole-Building Embodied Carbon Workflow.” Building Enclosure. 30 June 2021. Web. 9 Feb. 2022. <https://www.buildingenclosureonline.com/blogs/14-the-be-blog/post/89961-defining-a-whole-building-embodied-carbon-workflow>
35Alter, Lloyd. “New Tool Calculates Full Life Cycle Carbon Emissions for Passive House.” Treehugger. 25 Jan 2022. Web. 9 Feb 2022. <https://www.treehugger.com/tool-lifecycle-carbon-emissions-passive-house-5216866>
36International Living Future Institute and Passive House Institute. “Zero Energy & Passive House Certifications.” 2019. Web 22 Feb. 2022. <https://living-future.org/wp-content/uploads/2019/07/Zero_Energy_PassiveHouse_Crosswalk-1.pdf>
37Ibid.
38Resilient Design Institute. “What is Resilience?” Resilient Design. Web. 4 Feb. 2022. <https://www.resilientdesign.org/defining-resilient-design/>
39Roofing Contractors Association of British Columbia. “Template: ROOFTOP EQUIPMENT & WALKWAYS (BUR).” Web. 4 Feb. 2022. <https://rpm.rcabc.org/index.php/Template:ROOFTOP_EQUIPMENT_%26_WALKWAYS_(BUR>
40Green Roofs for Healthy Cities. “About Green Roofs.” Greenroofs.org. Web 10 Feb. 2022. <https://greenroofs.org/about-green-roofs>
41Simon, Matt. “Your Rooftop Garden Could Be a Solar-Powered Working Farm.” “Wired,” 3 Dec. 2021. Web. 17 Feb. 2022. <https://www.wired.com/story/your-rooftop-garden-could-be-a-solar-powered-working-farm/>
42Urban Green Council and The Nature Conservancy. “NYC’S SUSTAINABLE ROOF LAWS.” Urban Green Council. Dec. 2019. Web. 10 Feb. 2022. <https://www.urbangreencouncil.org/sites/default/files/sustainable_roof_laws_brief_final_12.11.19.pdf>
43Ibid.
44Javits Center. “Green Roof Tours.” Javits Center. Web 7 Feb. 2022. <https://javitscenter.com/sustainability/green-roof-tours/>
45Sokol, David. “Rooftop Farm Soars Above the City.” Architectural Record. 15 Dec. 2021. Web. 7 Feb. 2022. <https://www.architecturalrecord.com/articles/15429-rooftop-farm-soars-above-the-city>
46Designing Buildings. “Blue Roof.” 15 Dec. 2021. Web. 22 Feb. 2022. <https://www.designingbuildings.co.uk/wiki/Blue_roof>
47Livingroofs.org. “The blue green roof—helping cities cope with stormwater.” Web. 22 Feb. 2022. <https://livingroofs.org/introduction-types-green-roof/blue-green-roof-cities-stormwater/>
48SemperGreen USA. “Purple-Roof Case Study.” 12 Oct. 2021. Web. 22 Feb. 2022. <https://www.sempergreen.com/en/sempergreen-academy/webinar-innovation-for-future-proof-cities>